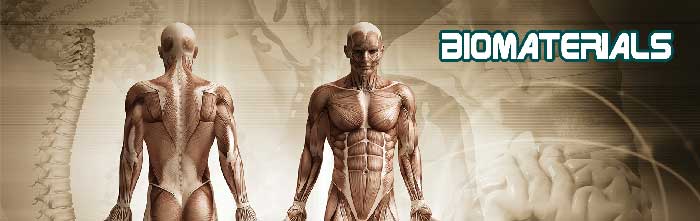
Biomaterials
It is possible to delimitate the study of biomaterials through a very general but coherent definition by Park and Lakes [1]. According to them a biomaterial can be defined as any material used in the manufacturing of devices to replace part or function of the body in a safe, reliable, economic, and physiologically acceptable way.
In order to satisfactorily perform the function of replacing, enlarging, or supporting a structure, the implant must mimic or match the characteristics of the tissue. The literature reports the existence of different types of bone grafts used in implants: autologous (derived from the same individual); homologous (from an individual of the same species of the receiver); and heterologous (when the species of the donor and the recipient are not the same – cattle, for example [2]).
Autologous grafts have certain drawbacks, for example: intervention in a healthy area of the patient’s body; donor area morbidity, higher recovery period; susceptibility to infections; and progressive and constant resorption [3]. Thus, implants in synthetic biomaterials such as ceramics and polymers have been widely developed and applied. According to Pereira, Buono and Zavaglia [4], the demand for biomaterials has grown from 5 to 15% every year.
Classification of biomaterials
According to Bath [5] and Santos [6], biomaterials can be classified into four classes, according to the compatibility they have with the surrounding tissues:
Biotolerant: Implant separated from the surrounding bone by a layer of soft tissue over the interface. No contact in the osteogenesis. The layer is induced by the implant release of monomers, ions, and/or corrosion products. Almost all synthetic polymers and most metals are this category.
Bioinert: Implants in direct contact with bone tissue, occurring involvement in the osteogenesis. However, there is no chemical reaction between the tissue and the implant. There is not, at least in amounts detectable by cells, the release of any component. Examples of bioinert biomaterials are: alumina, zirconia, titanium, tantalum, niobium, and carbon.
Bioactive: there is the interaction between the implant and the bone tissue, interfering directly in the osteogenesis. By chemical similarity, the mineral part of bone tissue binds to the implant, promoting osteoconduction. The main materials of this class are: Ca-phosphate, vitro-ceramic, and hydroxyapatite.
Bioresorbable: Materials that, after a certain period of time in contact with the tissues, end up by being degraded, solubilized, or phagocytosed by the body. They are of interest in clinical applications where it is inadvisable the reoperation to remove the implant. Representative of this class are tricalcium phosphate (TCP) and PLLA (poly-L-lactic acid).
Metallic biomaterials
Sometimes, metallic elements in their natural forms and in small quantities are tolerated by the body, such as iron (Fe) in red blood cells, cobalt (Co) in the synthesis of vitamin B12 [7], and in the cross-links of the elastin present in the aortic artery [1]. However, in large quantities most metals are not tolerated by the body.
Some metals are used as substitutes for hard tissues, for example, in total hip and knee replacements, plates and screws for fixation of fractures, column fixation devices, and dental implants because of their excellent mechanical properties and corrosion resistance [7]. According to Barbucci [8], a metal material used in the construction of orthopedic prostheses, osteosynthesis devices, and dental implants should have the following characteristics:
- Limit resistance equal to or greater than 800 MPa;
- Resistance to corrosion (local and general);
- Biocompatibility.
Biocompatibility is not an effect or a unique phenomenon. It refers to a range of processes involving different interaction mechanisms but interdependent concerning materials and tissue. It is the ability of the material to perform a specific function in the body without causing toxic or injurious effects on the biological systems [10].
These characteristics are so restrictive that only a small number of classes of metallic materials can be successfully used.
The first alloy specifically developed for the use in the human body was the “vanadium steel”, used for the manufacturing of plates and screws for bone fractures [1]. Among the metallic biomaterials, austenitic stainless steels of type 316 LVM, alloys Co-Cr-Mo, Co-Ni-Cr-Mo, pure titanium, and Ti-6Al-4V are the most widely used [9]. Some examples are shown in Figure 1.
Figure 1: Metallic devices of medical application: a) Femoral head; b) superior articulation knee; c) Mandibular joint plate.
In this work, we will address more specifically alloys Co and Ti, whose application in DMLS rapid prototyping in the medical field is more expressive.
Cobalt alloys
Cobalt alloys were originally proposed for surgical implants more than 70 years ago [11]. There are basically two types of Co alloys for medical application. The alloy Co-Cr-Mo for coating and the worked alloys Co-Ni-Cr-Mo [7]. The American Society for Testing and Materials (ASTM) lists four Co alloys that are recommended for the use in surgical implants: coating alloy Co-Cr-Mo (F75) and worked alloys Co-Cr-W-Ni (F90), Co-Ni-Cr-Mo (F562), and Co-Cr-Mo (F1537).
These alloys are a class of materials highly resistant to corrosion in physiological environments and to wear, outdoing the stainless steel [12]. Moreover, its superior resistance limit and fatigue resistance enable its application where long service life without the occurrence of fractures or stress/fatigue is required [1]. Many properties are originated from the crystallographic nature of cobalt, the booster effect of Cr and Mo, and the carbides formation of high hardness [13].
Such special characteristics have led to the use of these alloys in various medical applications, particularly in those that aim to replace the articular surfaces. Their properties of resistance to wear, corrosion, and low coefficient of friction are decisive in this choice.
An example of application is the Total Hip Arthroplasty (THA). The newer systems consist in a femoral rod attached to a modular head subject to coordination with the acetabular component [14]. This system is known as MoM (metal on metal) – in reference to the surface of contact – and is better than MoP (metal on polyethylene) systems, used in relation to the volumetric rate of wear and other parameters so far, as reported in [15]. Figure 2 shows an example of the MOP and MoM systems for THA.
Figure 2: On the left: MoP; on the right: MoM.
Another example of application of Co alloys, to which this work is directly related, is the total reconstruction of the temporomandibular joint. This type of prosthesis, known as Christensen prosthesis, has been in use for over 30 years and is characterized by the promotion of a stable, reliable, and predictable reconstruction, providing the reduction in the level of pain, improvement in the functionality, and higher degree of movement [16]. Figure 3 illustrates a temporomandibular prosthesis.
Figure 3: Temporomandibular prosthesis.
Titanium alloys
Discovered in 1791 by William Gregor, a British mineralogist [17], titanium – and more recently, its alloys – has been used for decades in the fixation of fractures and joint reconstruction as it meets the necessary requirements for biomedical applications, such as: wear resistance, biocompatibility, bioadhesion (induction of bone growth), modulus of elasticity (the closer to the human bone – 10-30 GPa -, the better), fatigue resistance, and good processability [18], [19].
Titanium has a hexagonal closed packed (hcp) structure relating to the alpha phase, which may undergo an allotropic change at 881º C for the body-centered cubic (bcc) structure known as the beta phase. The manipulation of these crystallographic characteristics by the addition of binders and thermomechanical processes allows the obtainment of alloys with various properties. [20]
The titanium classes that were initially introduced as biomaterials were ASTM F67 (commercially pure titanium in grades 1, 2, 3, and 4), ASTM F136 (alloy Ti-6Al-4V Extra Low Interstitial), and ASTM F1472 (alloy Ti-6Al 4W-standard) [21]. In these materials, some alloy elements stabilize the alpha phase while others stabilize the beta phase. One can stabilize the alpha phase by including aluminum, tin, and zirconium, whereas the beta phase can be stabilized with vanadium, molybdenum, niobium, chromium, iron, and manganese [13]. It is noted, therefore, that the alloy ASTM F136 is an example of stabilized alpha/beta alloy.
Part of wear resistance observed in titanium and its alloys is due to the formation of a passive oxide layer consisting primarily of TiO2 which adheres to the surface of the metal and protects it, in addition to being largely responsible for the biocompatibility of the material. [18] This layer may be obtained by anodic oxidation in acetic acid electrolyte and, when the presence of titanium in tissue adjacent to the implant is evaluated, it is substantially smaller in the anodized implant if compared to those without surface anodizing [22], [23].
Severe trauma in facial structure requires a large number of plates and screws, and the titanium implants are particularly suitable, [9] because of the properties cited in this review. There are plates and screws for the cranio-maxillofacial region in various configurations so that the doctor can adjust the system to the patient’s anatomy. Figure 4 is an example of application of titanium in fixation of cranio-maxillofacial fractures.
Figure 4: Plates for fixation of cranio-maxillofacial fractures.
Another application that demonstrates the versatility of the material occurs in the intramedullary rods for tibia closed and compound fracture [24], where the implant (Figure 5) is indicated for larger bone fractures and subjected to bigger mechanical stresses (tibia).
Figure 5: Tibial intramedullary shaft in Ti.
Ceramic Biomaterials
The development of ceramic materials for biomedical applications focuses mainly in the areas of orthopedics and dentistry [25]. It is a class of materials that includes several features of biomaterials. It has representatives of the bio-inert, bioresorbable, bioactive, and porous classes for tissue growth [26].
The potential of the ceramics as biomaterials comes from its similarity to the physiological environment, due to its basic constitution of ions that are also found in physiological environment (calcium, potassium, magnesium, sodium, etc.) and others whose toxicity is very limited (zirconium and titanium) [27].
The bio-inert ceramics are more representative in the compounds of alumina (Al2O3), zirconia (ZrO2), and zirconia stabilized with yttrium oxide (ZrO2 (Y2O3)). Their ability to not react with the surrounding tissue, corrosion resistance, high wear resistance, and high mechanical resistance are essential features in their use as articulating surfaces subjected to loads and friction [26], [28].
At the heart of bioactive and bioresorbable ceramics, one can find compounds such as hydroxyapatite (HA), calcium phosphates, especially the β-tricalcium phosphate (β-TCP), as well as the bioglasses and glass-ceramics, whose composition is comprised of an extensive range of oxides (SiO2, P2O5, CaO, CaF2, Na2O, Al2O3, Ta2O5, and TiO2, among others.) [28].
References
[1-8] Park, J; Lakes, R. S. “Biomaterials – An Introduction”. Springer. 3ª edição. 2007. p. 2.
[2-9] Gália, C. A; et al. “Uso de enxerto homólogo e heterólogo em diáfise femoral de ratos: comparação entre enxerto ósseo congelado e liofilizado“. Revista Brasileira de Ortopedia e Traumatologia. Março 2005.
[3-10] Marzola, C; Toledo Filho, J. L. “Fundamentos de Cirurgia Buco Maxilo Facial – Os implantes de materiais aloplásticos”. Capitulo X.
[4-11] Pereira, M. M; Buono, V. T. L; Zavaglia, C. A. C. “Materiais metálicos: ciência e aplicação como biomateriais. In: Oréfice, R. A; Pereira, M. M; Mansur, H. S. “Biomateriais: fundamentos e aplicações”. Rio de Janeiro. Cultura Médica, 2006, cap. 2, p. 39-58.
[5-12] Bath, S. V. “Biomaterials”. Narosa Publishing House. Nova Dheli, Índia. 2002. p.181
[6-13] Santos, L.A. “Desenvolvimento de fosfato de cálcio reforçado por fibras para uso na área médico-odontológica.” Tese de Doutorado. Universidade Estadual de Campinas. 2002.
[7-14] Wong, J. Y; Bronzinho, J. D. “Biomaterials”. Taylor e Francis Group. LLC. 2007. p. 1-1.
[8-15] Barbucci, R. “Integrated Biomateriais Science”. Kluwer Academic / Plenum Publishers. Nova Iorque.2002. cap. 6. p. 289-290.
[9-16] Bertol, L. S. “Contribuição ao estudo da prototipagem rápida, digitalização tridimensional e seleção de materiais no design de implantes personalizados”. Dissertação de Mestrado. Universidade Federal do Rio Grande do Sul. Porto Alegre. 2008.
[10-17] Donachie M. “Metals Handbook Desk Edition”. 2ª edição, editada por Davis, J. R. ASM International. 1998.
[11-18] Disegi, J. A; Kennedy, R. L; Pilliar, R. “Cobalt-Base Alloys for Biomedical Application”. ASTM – STP 1365. 1999.
[12-19] Shi, D. “Introduction to Biomaterials”. Tsinghua University Press, World Scientific. 2006. p – 123.
[13-20] Marti, A. “Cobalt-base alloys used in bone surgery”. Injury – International Journal of the care of the Injured. 2000 (31).
[14-21] Wnek, G. E; Bowlin, G. L. “Encyclopedia of Biomaterials and Biomedical Engineering”. Vol 2. 2ª edição. Informa Healthcare. 2008.
[15-22] Sieber, H. P; Rieker, C. B; Köttig, P. “Analysis of 118 second-generation metal-on-metal retrieved hip implants”. The Journal of Bone & Joint Surgery (Br). 1998;80-B:46-50.
[16-23] Garret, W. R; Abbey, P. A; Christensen, R. “Temporomandibular joint reconstruction with a custom total temporomandibular joint prosthesis: Use in the multiply operated patient”. A reprint from Surgical Technology International VI.
[17-24] Leyens, C; Manfred, P. “Titanium and Titanium alloys: fundamentals and applications”. Wiley-VCH. First edition. 2003. p – 1.
[18-25] Yaszemski, M. J; Tantrolo, D. J; Lewandrowski, K; Hasirci, V; Altobelli, D. E; Wise, D. L. “Biomaterials in orthopedics”. Marcel Dekker Inc. 2004. p – 2.
[19-26] Lütjering, G; Williams, J. C. “Titanium – Engineering Materials and Processes. Springer-Verlag. Second edition. 2003. p – 399.
[20-27] Brown, S. A; Lemons, J. E. “Medical applications of titanium and its alloys: the material and biological issues”. Proceedings of a symposium held in 1994 in Phoenix, Arizona. STP 1272. ASTM. 1996.
[21-28] Brunette, D. M; Tengvall, P; Textor, M; Thomsen, P. “Titanium in medicine: material science, surface science, engineering, biological responses and medical applications”. Springer-Verlag. 2001. p – 28.
[22-29] Larsson, C; Thomsen, P; Aronsson, B. O; Rodahl, M; Lausmaa, J; Kasemo, B; Ericson, L. E. “Bone response to surface-modified titanium implants: studies on the early tissue response to machined and electropolished implants with different oxide thicknesses”. Biomaterias 17 (1996) 605-616. Elsevier .
[23-30] Jorgenson D. S; Centeno, J. A; Mayer M. H; Topper, M.J; Nossov, P. C; Mullick, F. G; Manson, P. N. “Biologic response to passive dissolution of titanium craniofacial microplates”. Biomaterials 20 (1999) 675-682. Elsevier.
[24-31] Zimmer Sirus® – Intramedullary Nail System. Zimmer, 2007.
[25-32] Shakelford, J. F. “Bioceramics – Advanced ceramics; v. 1”. Gordon and Breach Science Publishers, 1999. p – 5.
[26-33] Hench, L. L. “Bioceramics: From concept to Clinic”. J. Am. Ceram. Soc., 74 (7) 1487-510 (1991).
[27-34] Hench, L. L; Wilson, J. “An introduction to Bioceramics”. World Scientific Publishing Co, 1993. p – 25.
[28-35] Bubok, V. A. “Bioceramics – Yesterday, Today, Tomorrow”. Powder Metallurgy and Metal Ceramics, Vol. 39, Nos. 7-8, 2000.
Customizable prostheses
Cranio-maxillofacial reconstruction via rapid prototyping
Rapid prototyping is the technique of parts manufacturing by the additive method. A 3D model created in a CAD system is sectioned into 2D profiles which are then constructed by rapid prototyping equipment layer by layer. Several techniques are available today, such as: stereolithography (SLA), Direct Metal Laser Sintering (DMLS), Laser Surface Melted (LSM), Fused Deposition Modeling (FDM), 3D printing (3DP) and Electron Beam Welding (EBW) [1].
In the first decade of use of the DMLS technique, from 1994 to 2004, it was dominated, at least in its commercial applications, by materials developed specifically for the DMLS process, namely with focus on improvements in the processability and properties acceptable for the most common applications. Nowadays, a wide range of alloys is available for use in DMLS, such as light alloys of titanium. Of special interest in medical area are the Ti-6Al-4V – titanium, aluminum, vanadium, and Ticp – commercially pure titanium. Both are characterized by their excellent mechanical properties, corrosion resistance, low specific weight, and especially by its biocompatibility [4] [5].
Traditionally, rapid prototyping (RP) is used in industry and engineering as a means to produce reliable prototypes in a short time, which brings gains in cost [1]. Recently, RP has extended its application beyond engineering products. Its use in the medical field to the manufacturing of custom implants and prostheses, the study of anatomy, and surgical planning include important research fields [2]. The appeal concerning the medical field occurs facing significant obstacles, such as the fact that a case is never equal to each other – which is why standardization in the production of implants is not advantageous – and issues regarding the obscure points of the region of surgery make surgical planning difficult [3].
Among the congenital defects, craniofacial anomalies (CFA) are a group highly diverse and complex that affect a significant proportion of people in the world [6].
Besides the cases of congenital deformities, there are craniofacial defects acquired due to other disorders – tumors, for example. In the last four decades, a growing volume of cases of facial trauma has also been observed, which is closely connected with the increase of car accidents and urban violence. [7]
In all cases, the cranio-maxillofacial rehabilitation is part of the process of reintegration of patients into the society and the promotion of well-being.
Rapid prototyping in skull and face reconstruction
The conventional means of manufacturing an implant for repair of cranial defect consists in the direct modeling of the implant in the surgical site during surgery. The printing is done in wax and subsequently used in the manufacturing of a biomaterial mold [8]. At the end of the 1980’s, the results of the first research which tried to find a way to produce a physical model directly from a digital three-dimensional model appeared [9].
Rapid prototyping is a manufacturing method layer by layer that can produce complex geometry from a CAD model [10].
Its use in medical applications has revolutionized the planning of complex surgeries through the construction of preoperative anatomical models that allow the medical staff to have a critical evaluation of each particular case [11]. It has also received great emphasis on its use in the customized manufacturing of implants for the reconstruction of the skull and facial flaws [12], [8], [13], [14], [15].
In the construction of customized implants, the input of the patient´s data is required. The data are obtained from CT or NMRI in 2D transverse slices in DICOM format, whose thickness is determined on the equipment when obtaining the images. The number of slices is what determines the image resolution and therefore the 3D model. The 2D slices are transferred onto the medical modeling software where it is possible to adjust the threshold signal in order to obtain, after interpolation, a 3D representation of the bone structure [11], [13].
After the obtainment of the model, it can be handled in CAD software for the modeling of the implant that will repair the bone defect.
The patterned solid is then converted into the STL format and can be prototyped by any rapid prototyping techniques.
Recent applications, materials, and case reports
In the cranio-maxillofacial repair area, several cases of successful application of 3D modeling technologies and rapid prototyping to have customized implants have been reported.
Bertol et al. [14] reported the use of tomography images in getting a 3D virtual model for virtual resection procedure of part of the jaw affected by a tumor and subsequent implant design to replace the affected region by the technique of mirroring by plane of symmetry, where the healthy portion of the jaw is mirrored and used in the reconstruction of the removed region.
Figure 7: Obtainment process of the 3D model and implant (on the left). Implant built by DMLS (on the right) [14].
The implant was built in titanium (Ti-6Al-4V) by using the technique of Direct Metal Laser Sintering (DMLS). In this process, the powdered metal is melted into a solid part through the local melting provided by a laser beam of high energy directed by computer according to the design of the part.
After the manufacturing of the implant, it was scanned by the 3D scanning technique for the dimensional comparison with the virtual model. The differences were not bigger than 0.05 mm, which demonstrates the accuracy of the method.
Drstvensek et al [16] also reported successful cases that demonstrate the great potential of rapid prototyping in the medical field. In one reported case, a severe facial asymmetry (hemifacial microsomia) was treated. The used methodology was similar to that used by Bertol et al. 3D models were obtained from CT images and the implant was developed in virtual environment by mirroring the unaffected part.
Figure 8: Virtual model of the area to be constructed (on the left); Implant manufactured by DMLS [47].
In another case reported by Drstvensek et al [16], it was used the same techniques in the manufacturing of a skull implant and posterior 3D scanning for dimensional control. In comparison with the virtual model, the implant showed variations of 0.8 to 1.0 mm in some regions. According to the author, due to the fact that the region in question is not located in a critical part, the implant was approved and successfully implemented. The prototyping technique used in both cases was DMLS and the material was Ti-6Al-4V.
Figure 9: Prosthesis manufactured by DMLS (on the left). Result of the virtual geometric inspection (on the right).
Recently, several studies have focused on the properties association in the manufacturing of implants. This occurs, for example, in the combination of mechanical resistance properties and titanium corrosion with the bioactivity of ceramics such as HA and α-TCP.
Ning and Zhou [17], [18] evaluated the bioactivity in vitro and in vivo of biocomposite fabricated from HA and Ti powders by the powder metallurgical method. Mixtures in various proportions of HA/Ti were mixed and synthesized at 1200° C. The results show that the composites with the highest Ti content have the ability to induce nucleation and growth of apatite on their surface, which provide bone growth and make the metal/ceramic biocomposites candidates for bone replacement.
Kim et al deposited dense and uniform films of HA and FHA (hydroxyapatite-fluoro) with thickness ~ 5 μm in titanium substrates using the sol-gel technique. The substrate presented favorable growth and proliferation of osteoblasts, increasing the activity and function of the substrate.
Along the same line of work, Bertol [9] reported two cases with the association of Ti and α-TCP. A customized implant was obtained by manual molding on the physical model of the patient obtained from tomographic images (orbital floor), whereas the other was machined according to the virtual design also obtained from tomographic images (mandible). In both cases, the implants were coated with α-TCP and successful implemented.
Figure 10:
Not only the titanium but also the Co alloys have been using ceramics composites in order to improve some of its properties. Yen et al report the electrolytic coating of an alloy Co-Cr-Mo with ZrO2 to a hip prothesis. In this case, the application of the coating reduced the wear of the metallic component against the polymeric component and decreased the coefficient of friction between the surfaces.
References
[1] Khan, S. F.; Dalgarno K. W. “Design of customized Medical Implants by Layered Manufacturing”. School of Mechanical and Systems Engineering. NC University – UK.
[2] Ma, D; Lin F; Chua C. K. “Rapid Prototyping Applications in Medicine. Part 1: NURBS-Based Volume Modelling”. The International Journal of Advanced Manufacturing Technology. Springer-Verlag London Limited. 2001
[3] Lima, B. C. “Engenharia Reversa e Prototipagem Rápida: Estudo de Casos”. pp 15-16. 2003.
[4] Shellabear, M; Nyrhilä, O. “DMLS – Development History and State of the Art”. LANE, Erlanger. 2004.
[5] Shellabear, M; Nyrhilä, O. “Advances in Materials and Properties of Direct Metal Laser-Syntered Parts”. LANE, Erlanger. 2004.
[6] Molleó, I. L. “Anomalias Craniofaciais, Genética e Saúde Pública: contribuições para o reconhecimento da situação atual da assistência no Sistema Único de Saúde”. Unicamp. 2004.
[7] Lima Silva, J. J. de; Lima, A. A. A. S.; Torres, S. M. “Fraturas de face: análise de 105 casos”. Revista Brasileira de Cirurgia Craniomaxilofacial / Associação Brasileira de Cirurgia Crânio-maxilo-facial. – Vol.12, n.1 (Jan.2009).
[8] Gopakumar, S. “RP in medicine: a case study in cranial reconstructive surgery”. Rapid Prototyping Journal. Volume 10 · Number 3 · 2004 · pp. 207–211.
[9] Bertol, L. S. “Contribuição ao estudo da prototipagem rápida, digitalização tridimensional e seleção de materiais no design de implantes personalizados”. Dissertação de Mestrado. Universidade Federal do Rio Grande do Sul. Porto Alegre. 2008.
[10] Naber, H. “Advances in rapid prototyping technologies”, Materials and Manufacturing Conference, Metalex, Thailand. 1998.
[11] Gibson, I; Cheung, L. K; Chow, S. P; Cheung, W. L; Beh, S. L; Savalani, M; Lee, S. H. “The use of rapid prototyping to assist medical applications”. Rapid Prototyping Journal 12/1 (2006) 53–58.
[12] Hench, L. L. “Bioceramics: From concept to Clinic”. J. Am. Ceram. Soc., 74 (7) 1487-510 (1991).
[13] Wu, W; Shang, Y; Li, H; Wang, W. “Fabrication of repairing skull bone defects based on the rapid prototyping”. Journal of Bioactive and Compatible Polymers, Vol. 24 — May 2009.
[14] Bertol, L. S; Junior, W. K; Silva, F. P. d. ; Aumund-Kopp, C. “Medical design: Direct metal laser sintering of Ti-6Al-4V”. Materials and Design (2010), doi: 10.1016/j.matdes.2010.02.050.
[15] Oliveira, R. S. d; Brigato, R; Madureira, J. F. G; Cruz, A. A. V; Filho, F. V. d. M; Alonso, N; Machado, H. R. “Reconstruction of a large complex skull defect in a child: a case report and literature review”. Childs Nerv Syst (2007) 23:1097–1102.
[16] Drstvensek, I; Hren, N. I; Strojnik, T; Brajlih, T; Valentan, B; Pogacar, V; Hartner, T. Z. “ Applications of Rapid Prototyping in Cranio-Maxilofacial Surgery Procedures”. Internacional Journal of Biology and Biomedical Engineering. Issue 1, volume 2, 2008.
[17] Ning, C. Q; Zhou, Y. “In vitro bioactivity of a biocomposite fabricated from HA and Ti powders by powder metallurgy method. Biomaterials 23 (2002) 2909–2915.
[18-49] Ning, C. Q; Zhou, Y. “Correlations between the in vitro and in vivo bioactivity of the Ti/HA composites fabricated by a powder metallurgy method”. Acta Biomaterialia 4 (2008) 1944–1952.